McAlear Lab Makes Fundamental Discovery About How Cells Control Genes
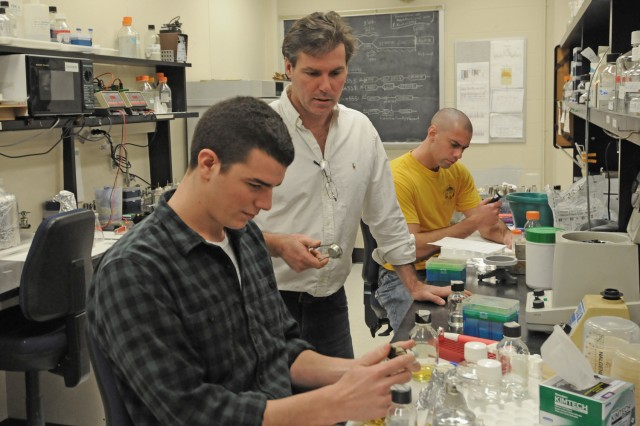
(Story contributed by Jim H. Smith)
A recent discovery made by Michael McAlear, chair and associate professor of molecular biology and biochemistry, and four of his students helps explain the function of ribosomes and sheds light on genetic processes scientists have been trying to decode for more than half a century. A paper authored by McAlear and his laboratory team, describing the discovery, was published last month in the distinguished journal BMC Genomics.
The discovery resulted from long-term research into the regulation of ribosomes, often described as molecular “machines,” the organelles of all living cells in which protein synthesis occurs. Guided by genetic “blueprints” delivered to them by messenger RNA, ribosomes gather amino acids, the building blocks of protein, and assemble them into polypeptides, the intricate chains of amino acids that, depending upon their arrangement, form the unique protein characteristics required for specific biological functions.
Scientists have known about ribosomes and their essential function for a long time. Cell biologist George Palade was awarded the 1974 Nobel Prize in Physiology and Medicine for discovering them in 1955. But while scientists have understood what ribosomes are and what they do, they’ve spent the last half century trying to decipher the complex array of metabolic processes that play a role in the production of new proteins. It is one thing to know what ribosomes do, quite another to know how they do it.
“Basically, genes are recipes,” McAlear says. “By switching them on and off, in an orderly pattern, they instruct ribosomes on how to synthesize polypeptides. They define the structure of an organism. The question is, how do genes get turned on and off?”
The research that has preoccupied McAlear’s lab for several years evolved from a landmark discovery made in 1961 by François Jacob and Jacques Monod. The French biologists showed how multiple genes in the bacterium E. coli are arranged in precise orders–known as operons–which, governed by a single regulatory signal, work together in response to shifting environmental conditions.
Operons are most prevalent in prokaryotes, organisms whose cells lack a nucleus. Only a few eukaryotes – the large group of organisms whose cells contain nuclei – have operons. However, the principle described by Jacob and Monod and their colleagues inspired McAlear and his students to look at other factors controlling genes in eukaryotes. The subject of their research was Saccharomyces cerevisiae (S. cerevisiae), the most useful of all the yeasts, the one that has been used for centuries in baking and the fermentation process that yields alcohol. “In eukaryotes, having a nucleus allow for the controls of genes in more complex ways,” says McAlear.
Using sophisticated computational analysis, McAlear and four students – Ph.D. candidate James Arnone, Jeffrey Arace ’12, Adam Robbins-Pianka BA ’08, MA ’10 and Sara Kass-Gergi ’12 – discovered 200 genes in S. cerevisiae that had characteristics in common distinguishing them from the yeast’s nearly 6,000 other genes. Two special attributes made the 200 genes significant. First, unlike the apparently random arrangement of most genes, many of these were neatly placed on the yeast’s chromosomes as immediately adjacent pairs. And second, they were turned on when a cell needed to build more ribosomes.
Similar to the operons in prokaryotes, the gene pairs were special arrangements linking their physical position and their activity. “We found that, as a whole, adjacent genes are more tightly co-regulated than unpaired genes,” says McAlear. Further investigation revealed that the pairing phenomenon persisted across other, divergent forms of fungi. And then, as McAlear’s team looked at the gene positions of ribosome-related genes across a strikingly diverse set of eukarotes, well beyond the family of fungi, they also found significant levels of gene pairing in those species, including humans.
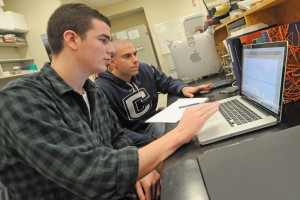
“The evidence was screaming at us that something significant was going on,” McAlear says. Indeed, something was. As the team explored the phenomenon, they quickly came to understand that the paired genes worked in concert, one reliant upon the other. Further, one gene in the pair can be dominant, and it can drive the expression of its immediate neighbor. If anything comes between the pair – if, for instance, another gene is artificially inserted in the sequence – they are rendered incapable of exerting the co-regulatory influence.
The discovery, McAlear says, is important in several respects. “The significant of understanding how organisms ‘use’ their genes appropriately is as important as ever given that advancing, massively parallel DNA sequencing technologies continue to provide an ever-increasing catalog of new and diverse genomes.” Noting that his team has been dedicated to understanding how ribosome biogenesis is regulated, he asserts that “We’ve made a fundamental discovery. We’ve taken a big step toward understanding how cells control their genes.”
While it’s much too early to predict just what impact the research will have on the rapidly evolving field of molecular biology, McAlear notes that it has some important medical implications. “The target of many antibiotics is ribosomes,” he says. “If this research led to treatments that could temporarily stop the biosynthesis of ribosomes, it could significantly help the development of new antibiotics to treat many diseases. Also, ribosome production that has gone awry is a central activity in the development of cancer. Suppose there were a treatment to easily disrupt the signaling of related gene pairs that cells need to make ribosomes, and proteins required for cell division.”